I. Introduction
The shearing of confined granular materials may look like a rather simple topic. The geometry of such a system is straightforward (Fig. 1a): it consists of a layer of granular (or at least, divided) matter trapped under two, possibly rough, rigid walls, subjected to a certain normal stress, and to a certain shearing through an imposed tangential velocity on one or both walls. In this paper, we pass over any wall-slip effect and any mechanical coupling with a suspending fluid (although those topics are of some importance in the applications that will soon be presented), thus focusing our attention on the response of the central layer to imposed shearing. Under these loading conditions, the case of a granular material constituted of rigid, frictional, quasi-monodisperse, circular or spherical grains, can be considered as solved under the celebrated “µ(I)” framework (1,2), where µ is the shear resistance expressed as a friction coefficient, and I = γd˙ p σ n /ρ is the inertial number of the flow. As we demonstrate in this paper, however, relaxing some of these assumptions severely complicates the problem. We may wonder, indeed, to what extent the existing theories and methods can deal with grains of complex shapes, with deformable grains, with strongly polydisperse grains populations, and with more complex interactions among grains and between grains and their environment. Current research on these topics is driven by many fields of application but we will focus here on two specific and related classes of problems: the tribological three-body contact, and the mechanics of seismic faults.
Tribology is the science of friction and wear in solid contacts, whether they be dry or lubricated. Since the pioneering works of Godet (3), we know that friction is not an intrinsic property of the materials but rather an emerging phenomenon with multiple causes, and that a better understanding of dry contacts requires the concept of a “third body” (Fig. 1b).
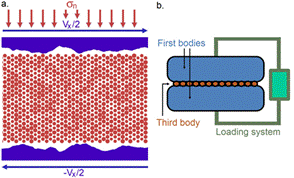
Figure 1: Conceptualization of the problem: a. General configuration of a confined sheared granular flow under a normal stress σ n and a relative velocity V x ; b. The tribological triplet, including the three tribological scales.
This third body is a layer of solid matter trapped within the contact interface, originating from the degradation of the contacting surfaces or from external material, which transmits the normal load and accommodates the possible sliding between the two “first bodies”. Countless experimental pieces of evidence (4-6) point to the fact that, except in some idealized lab conditions, dry contacts with a sufficient sliding history always fit in this “three bodies” model. Hence, first bodies are rarely in actual contact, and dry friction should mostly be considered as the result of the rheology of the (generally) nanometric to micrometric third body layer submitted to shearing. Third bodies are very diverse in thickness, chemical composition, visual aspect, and mechanical behaviour (Fig. 2), depending on the materials in contact and on a large number of other factors (including the plasticity/fracture response of the first bodies, the dynamics of the loading system, the contact load and velocity, the temperature, the chemical interactions with the environment, the contact history, etc.). Driven by industrial applications (including energy efficiency, since it is estimated that onefifth of world energy consumption is dedicated to overcoming friction, (7)), these systems are at the core of the tribological literature and are investigated with observational, experimental, numerical and theoretical tools (8-10). A specific and particularly interesting case of such tribosystems, although studied in a completely different scientific community, is that of seismic faults. These faults naturally exist in many locations on Earth (e.g. at the boundaries of tectonic plates, but elsewhere as well), and consist of localized, thin, somewhat planar, zones of weakness in the lithosphere, which accommodate sliding between rigid-like bodies of rocks. They span several spatial scales (from hundreds of kilometres down to less than a meter), and also contain a third body called “fault gouge” in the geological terminology (Fig. 2). An immense body of work focuses on the contact instabilities in these tribosystems, also called earthquakes (11). Obviously, a part of the answer lies in the rheological response of the gouge layer to shearing, and it is indeed the topic of an abundant geophysical literature. Apart from the discrete numerical approaches described later in this paper, several multiphysical continuum-based approaches are worth mentioning (12-14). The reader interested in this specific topic is referred to (15) and numerous references therein.
II. State of the art and recent numerical findings i Numerical tools
Can granular physics help on these topics? If we accept the representation of the third body, at least to a first order, as a divided, discontinuous solid material, the answer to this question is certainly positive. We can thus conceptualize the third body (or gouge) layer as a confined sheared granular material, and adopt the representation of Fig. 1. Discrete Element Modelling (DEM) has been widely used in this framework to investigate the rheological response of sheared layers under confinement (16-20), as well as the shearing of granular gouge (21-23). It is useful to recall here that DEM is a popular numerical framework in granular mechanics, into which the individual motions of all the (generally circular or spherical) grains composing a granular sample are computed based on Newtonian dynamics and on forces arising from interparticle (generally frictional) contacts. Compared to more common, continuum based, computational methods in mechanics (such as the Finite Element Method, FEM), DEM presents several advantages

Figure 2 SEM views of tribological third bodies. a: Metal-metal opened contact with evidence of granular and plastic accommodation regimes (27); b: Same system, evidence of compacted and agglomerated third body; c: Mineral grains of granular gouge in a rock sliding experiment (65); d: Evidence of gouge melting in a rock sliding experiment (65).
when applied to third bodies: it offers a large kinematic freedom which allows the representation of sheared flow in the presence of discontinuities; it does not rely on a mesh, which makes it robust up to an arbitrary strain level; and it does not require one to choose a predefined constitutive law for the solid domain to be simulated. For these reasons, several authors achieved notable success in understanding some characteristics of third body flows using this numerical tool (16-20). It should be noted, however, that most of these studies assumed circular/spherical grains and a very narrow particle size distribution (PSD), mostly for reasons linked in one way or another to the computational cost. They thus remained rather idealized case studies.
Indeed, despite these early achievements, we must point out an inherent limitation of DEM in this framework: the assumption of perfect rigidity for the grains (only mildly degraded by the numerical stiffness used for the computation of repulsive contact forces). This assumption is valid for a large majority of DEM applications, where the stiffness of the material composing the grains is several orders of magnitude larger than the typical stress they are submitted to. This is true, to a certain extent, for rock gouges in the shallower portions of faults. In the deepest areas of the lithosphere (e.g. beyond depths of 10-15 km), average stress levels can reach several hundreds of MPa, and local contact stresses between grains can therefore reach several GPa. Since the typical Young’s modulus of Crustal rocks is ∼ 10 − 100 GPa, we can reach strain levels of several %, and the assumption of grains rigidity becomes questionable. In industrial tribological contacts, average contact stresses are often well beyond the GPa, and local stress concentrations within contacts are expected to be much higher, which may bring us well above the elastic limit of the materials composing the third body. Under such conditions, it is likely that purely granular rheology, relying mostly on the steric exclusion between rigid bodies, might not be able to represent all classes of third body flows, especially the most plastic and agglomerated ones. An alternative approach would be to allow the grains to deform, which takes us out of the strict framework of DEM. Several numerical approaches have been proposed in related fields, notably that of powder compaction (24-27). These developments were also encouraged by a recent, growing interest of the granular experimental community in the field of deformable grains (28-32). For the remainder of this section, most of the examples were treated with the Multibody Meshfree Approach implemented in the open source code MELODY (33,34). This approach is based on a discretization of the domain covered by each grain using a certain number of field nodes, which carry the degrees of freedom in displacement. The displacement field is interpolated in each grain between the field nodes using shape functions, very much in the manner of FEM, but with Moving Least Square shape functions which do not rely on a mesh and are therefore much more robust to very large strains. A weak formulation of the continuum mechanics equation is then derived and integrated in time using an adaptive explicit solver. Contacts between the highly deformable bodies are dealt with using a robust two-pass node-to-segment algorithm. Thanks to this approach, MELODY can also handle rigid grains with arbitrary shapes in the DEM framework.
ii. An unexpected variety of kinematic regimes
It was indeed shown in (26) that allowing grains to deform made it possible to simulate confined sheared flows with a much wider variety of accommodation regimes. In particular, by varying systematically the stiffness of the grains, their cohesion (i.e. the strength per unit area of the interparticle contacts), and their material viscous damping, the relative motion between the two walls could be accommodated in many ways (Fig. 3a-d). The tendency of the grains to form agglomerates when sufficiently soft and cohesive, in particular, led to a reduction of the resulting friction coefficient of the interface by promoting rolling kinematics (Fig. 3c), which are much more energetically favourable than granular shearing (36). In some other cases, soft and cohesive grains were able, in contrast, to selforganize in force pillars and to optimize their collective resistance to shearing, thereby maximizing the interface friction coefficient (Fig. 3d). These observations were further confirmed in (37), where the effect of cohesion between grains on rheology and its relation to friction was studied in more details and where the causes and consequences of agglomeration were analysed. Meanwhile, in the field of seismic fault gouges, the focus of some recent studies was put on the shapes and interaction laws of rigid grains in a classical DEM framework. In (38), the effect of an initial cohesion of the mineral angular grains composing the gouge (because of physico-chemical cementation in the interseismic period) was studied, and its consequences on the frictional response of a seismic fault in the initial stages of a seismic sliding were observed. It appeared that, starting from a low cementation and a classical Couette flow regime (as expected for frictional grains), an increase in cohesion led to the appearance of oblique shear bands, also known in the geological literature as Riedel bands, and to the formation of rigid cohesive agglomerates of grains (Fig. 3k). In the case of very high initial cementation, the seismic sliding occurred by fault-parallel fracturing and extreme localization of the shear (Fig. 3l). The fault gouge simulated in (39) relied on a different representation of this cementation, under the form of a filling of the whole pore space by a matrix of very fine, conforming polygonal grains. These models led to even more various accommodation regimes with complex geometries, including the activation and deactivation of bulk shearing, primary and secondary Riedel bands, and finally the development of fault-parallel shear bands (Fig. 3n).
In (40), an additional degree of complexity was added in order to simulate the effects of shear heating on fault rheology. Indeed, due to the large normal stresses present in the deeper areas of seismic faults, the relatively high sliding velocity (up to sev eral m/s), the poor thermal conductivity of rocks, and the natural tendency of granular gouges to localize shear in narrow bands, large temperature elevations occur in the gouge layer. Under the right circumstances this can lead to gouge melting, and this has been widely documented in the field and in the lab (41-43). In (40), melt was numerically introduced in the granular gouge using frictionless, quasi-incompressible, very soft viscoelastic grains. It was observed that the presence of these molten grains led to a progressive localization of the shearing and to a non-linear decrease of the fault friction from a purely granular to a purely viscous regime, thereby finally reducing the amount of heat production through complex feedbacks (Fig. 3g-h). It is generally believed that water is present in deep crustal rock, although not in the form we are used to at the earth’s surface. Under several tens or hundreds of MPa, water is indeed physically or chemically adsorbed on any available surface or interface, and its effect on melting is therefore essentially physico-chemical. Its main consequence is a reduction of the melting point of minerals with respect to the completely dry case (44). The complex topic of the rheology of mixtures of soft and hard grains was also tackled in (45), showing interesting intermediate regimes where hard inclusions seem to nucleate short-lived shear localization between the soft grains, thereby temporarily creating rotating agglomerates without the presence of any dilatancy (Fig. 3e-f). As we see, the traditional picture of the sheared flow of circular quasi-monodisperse frictional rigid grains gets much richer, but also much more complicated, when one or several of these assumptions are relaxed. The reader can refer to the different papers cited in this survey to find more details about this complexity. The remainder of this paper is devoted to the presentation of several challenges in this field of study which are likely to be at the core of future works in the granular, tribological, and geophysical communities in the coming years.

Figure 3 Overview of a variety of kinematic regimes in confined sheared granular flows. a: Force chains (hard grains) (35); b: Plastic flow (soft and mildly cohesive grains) (35); c: Agglomerated rolling flow (soft cohesive grains) (35); d: Force pillars (critically soft and cohesive grains) (35); e: Hard clusters in a mixture of 40% soft and 60% hard grains (45) (colours code shear rate); f: Short-lived localized shear bands in a mixture of 80% soft and 20% hard grains (45) (colours code shear rate); g: Moderate localization in a granular gouge with 5% of molten grains (40) (colours code horizontal displacement); h: Strong localization and quasi-viscous flow in a granular gouge with 60% molten grains (40) (colours code horizontal displacement); i: Oblique fracture and horizontal localization in a mixture of a few large grains within a fine matrix (39) (colours code solid fraction); j: Rigid aggregated formation in a mixture of many large grains with porosity filled by a fine matrix (colours code solid fraction); k: Formation of cohesive aggregates and Riedel bands in a moderately cemented gouge (38) (colours code damage); l: Riedel bands and horizontal fracture in a highly cemented gouge (38) (colours code damage); m: Progressive destructuration of a large number of oblique bands towards bulk shearing (39) (colours code solid fraction); n: Activation of five primary shear bands in a fine matrix of conforming polygonal grains, only two of them being active in a later stage of shearing (39) (R refers to Riedel shear band, Y refers to boundary shear band, colours code solid fraction).
III. Some challenges
i. Experimental validation
A large number of experimental studies have been proposed by the scientific community to study confined sheared granular flows under various conditions. Quantitative statistics of seismic events were, for example, reproduced in the lab observing collections of photoelastic discs (46). Rock friction laws in the presence of a granular gouge have been derived from shearing experiments, including very fine second-order phenomena such as rate and state effects (47) and interface melting (41). Flows of grains with complex shapes (48) or with large deformability (31,32) are now increasingly reproduced and analysed in the lab. Countless rubbing experiments have been reported in the literature, involving a wide variety of materials. And yet, it does not seem excessive to write that dry friction is still a largely unsolved problem. In contrast with the lubricated contact, for which accurate models allow designing the sliding geometry and the lubricant rheology with great precision, no universal and predictive model exists for the quantitative prediction of dry friction and wear. Part of the explanation lies in the difficulty of relating local friction models with experimental observations and measurements. Regarding this difficulty, both models and experiments are to blame. Indeed, most quantitative theories of dry friction do not include the presence of a third body, and are therefore only applicable in very controlled lab conditions. Numerical models of contact interfaces including a third body are rare, and they remain quite idealized. Limitations arise from their limited scale (see section III.ii) and from their computational cost (see section III.iii), but more importantly from the limited set of physical assumptions on which they rely. We can mention two important bottlenecks:
The flow of third body occurs in a largely out-of-reach area: a mechanical contact. Of course, observation and even characterization (4, 5) of an interface can be performed post-mortem by various means, after the end of a tribological experiment. But what is observed and characterized is not, strictly speaking, a third body: it is merely a layer of wear debris. Indeed, mechanical and physical conditions drastically change when one stops a sliding experiment and opens a contact: removal of the normal stress, absence of a shear rate, temperature drop, exposition to the external atmosphere (oxidation, etc.), destruction of the possibly loose fabric, release of residual stresses, etc. The experimentalist thus only has a very partial view of what the third body was when it was active within the tribological contact. Despite the best efforts of the tribological community, there is today a lack of in situ third body characterization, essentially because this task appears extremely challenging from a practical point of view. Similar limitations exist in earthquake science, where field observation is generally limited to geologically exhumed and long inactive faults, and where lab experiments meet the same limitations as tribological tests.
In a mechanical contact, solid matter is submitted to extreme conditions. Consider for example a typical contact between metallic parts. The normal stress of a Hertzian contact between two centimetric spheres under common industrial loading can reach several GPa, a stress for which material characterization is indeed challenging. Now, if we consider in addition that a typical sliding speed is of the order of 1 m/s, and that a typical thickness for the third body layer is seldom above 10 µm, it means that a typical shear rate in this layer is at least of the order of 105 s−1. It also means that high temperature increase can be expected, at least locally. Theoretical models for the rheology of solid flows have been proposed and enriched with new physics, based for example on the kinetic theory or on the µ(I) rheology (49-52). But such theories are lacking in the range of loads, of temperatures and of shear rates that exist in the systems at stake here (not even mentioning the fact that the third body is a highly transformed and heterogeneous material, and therefore very different from that composing the first bodies). This leads to the somewhat paradoxical fact that the only way to characterize the interfacial layer of a friction test is to run a friction test. Without rheological laws established on independent characterization tests, the modeller is left with a lot to speculate upon when it comes to choosing the constitutive and contact laws to introduce in numerical models.
Apart from the constant improvement of in situ contact probing and of material characterization under extreme conditions, a possible way to indirectly link experiments and models is to rely on the concept of morphology. Tribologists with a large experience in post-mortem interface observation have indeed developed empirical knowledge which often allows them to infer meaningful tribological scenarios solely from the visual aspect of the contact observed, for example, through Scanning Electron Microscopy. This clearly indicates that a large part of the contact behaviour and rheology is coded in this visual aspect,whether in the form of certain shape properties or localization patterns of wear particles, or textural characteristics of the wear track at various scales, for example. Now, if third body models based on confined shearing of complex (e.g. cohesive, deformable, polydisperse) grains are able to reproduce such morphologies, it might indicate that they are getting more reliable and closer to the physical reality of the third body. A good way to build this missing link through morphology is to employ Machine Learning techniques, which are designed for such tasks where human intuition can hardly be written in explicit algorithms. Several recent (53) and ongoing (54) research projects have been following this avenue, and it is likely that this trend will increase in the years to come.
ii. Multiscale aspects
A very comprehensive conceptualization of tribological contacts is offered by the “tribological triplet” approach (55). This representation states that one cannot fully understand and predict the behavior of a contact without considering three different scales: the scale of the two bodies in contact, including their deformability and their response to the extreme loads applied to them in the vicinity of the contact (plasticity, fracturation, etc.); the (upper) scale of the loading system which contains these bodies, including its own actuators, stiffness, inertia, damping, etc.; and the (lower) scale of the third body layer, including all the complexities described in the previous section. Some orders of magnitude of typical tribological experiments are useful: a typical contact area is expressed in mm2, the typical length of a wear track is a few cm, and the typical thickness of the third body layer is hardly more than a few tens of µm, meaning that the typical volume of a third body can be approximated to about 10−9 m3. If we approximate the volume of an atom to 1 nm3, and the volume of a third body particle to 1 µm3, we must thus deal, in atomistic or discrete simulations, with a number of at least 109 to 1018 objects. Time scales are interesting as well. The typical duration of a friction test is a few minutes, and a wear test can extend to several hours. Typical time steps in atomistic or discrete simulations are of the order of 1 fs and 1 ns respectively, which means that we must expect between 1012 and 1020 time steps if such tests are to be entirely simulated. Considering that current models can deal with 105 to 107 objects during 106 to 108 time steps, it is clear that particle-based methods will never be used for the full simulation of tribological experiments. There is thus a need to establish a solid link between local particulate models (practically valid only on limited time and space scales) and the rest of the tribosystem. We can mention here three possible avenues to establish this link:

Figure 4 A model for laboratory earthquakes combining several relevant scales (59). A: Full view of the model with a precut deformable rock sample submitted to a confining lateral stress and placed between two deformable blocks (mimicking the compliance of the loading system), submitted to vertical compression; B: View of the mesh of the upper area of the system (notice the refined mesh in the vicinity of the contacting surfaces); C: Zoom on the initially intact interface, composed of rigid conforming polygons linked by a Cohesive Zone Model which can damage and break during sliding; D: Typical loading curve showing alternating stick (elastic increase of the load) and slip (sudden stress drop) periods of various durations and intensities, in good synchronicity with the average profile of damage in the fault; E: Snapshot of a small area of the contact showing the layer of granular gouge released in the interface, the damage distribution of the surfaces, and fracture patterns.
A link could be made through rheological laws. The concept of inertial number (1, 2) has proved a valuable tool for the quantitative description of sheared granular materials, albeit in a certain range of conditions only. Recent progress was made to extend this formalism to the case of cohesive materials (56), but this theoretical framework remains quite far from the complexity of tribological third bodies and of fault gouge, two domains of application for which it was not designed. This lack of applicability has two major bases, the first being that µ(I) rheologies rely on the concept of rigid grains, which provide a characteristic spatial scale on which shearing is accommodated between rigid objects. When the grains can easily deform (and thus accommodate shear in another manner than interparticle relative motion) and when the granular population is extremely polydisperse (such as in granular gouges with fractal particle size distributions), this characteristic scale disappears. The second reason is the fact that this rheology is mostly based on statements related to inertia, while tribological and fault-related confined flows are, rather counter-intuitively, quasi-static. Any computation of the inertial number of such flows, with appropriate guesses on the typical space scale, leads to I < 10−3, i.e. to dense quasi-static flows (41). This is mostly because of the very large confining stresses applied to these flows. Hence, any rheological law to be developed in these frameworks should be based on other criteria than inertia. As demonstrated in (35), future scaling laws able to discriminate between the extremely various kinematic regimes of shearing accommodation might be related to (i) the contact interaction between the grains, especially their cohesion, (ii) the constitutive behavior of the material composing the grains, including stiffness, ductility, and viscosity, (iii) shapes and sizes of the grains, and (iv) interactions between these three classes of properties. From rheology, friction laws might be developed and readily introduced in large scale models.
Another possible link between the different scales of a tribosystem could be built through numerical models containing physical insights from several scales at the same time. This is only partially possible in practice, essentially for computational reasons, and implies simplifying much of the physics considered at each scale. The purpose of such models is therefore not to describe each scale accurately, but to observe their interactions. In (57), for example, the focus was put on the interaction between the flow of an abrasive granular layer of debris in an interface, the subsequent fields of velocity and pressure in this flow, the wear rate of the surfaces of the first bodies confining this flow, and the feedback of this wear on the topographical evolution of these surfaces and therefore on the flow patterns. Hence, it used a Generalized Reynolds Equation (58) combined with a non-Newtonian rheology (of the Hershel-Buckley type) for the granular layer in order to derive the kinematic fields of the third body flow, which was then combined with an Archard-like wear law enriched with the statistical properties of the grains’ morphologies to predict the rate of wear, resolved in space and time. This model successfully predicted the wear rate and the wear profile after a completely independent calibration. It follows therefore that even highly simplified laws can be used if carefully chosen and calibrated, and coupled effectively. This model, however, can hardly be extended directly to other configurations than that for which it was designed. In a different framework, the fault model presented in (59) offers a direct coupling between the compliance of the loading system, the deformability of the contacting bodies, the degradation of the two surfaces in contact, the emission of granular gouge in the interface, and the subsequent modification of its rheology (Fig. 4). Based on a continuumdiscrete direct coupling implemented in MELODY, this model aims to reproduce numerically the main features of laboratory earthquake experiments (60), and indeed it succeeds in spontaneously nucleating typical seismic instabilities and stick-slip patterns. These patterns require both the compliance of the two contacting bodies and an interface prone to frictional weakening. While highly simplified in the representation of each scale, the model succeeds in reproducing these dynamics because it contains the right physical ingredients both in the continuum and discrete zones.
A last class of multiscale coupling is that related to REV-scale discrete simulations embedded as an alternative to constitutive laws in systemscale continuum-based simulations, as developed in the field of soil mechanics and geotechnical engineering (e.g. DEM×FEM, DEM×MPM, etc. (61, 62)). This interesting class of models is, to our best knowledge, unused in tribology and in fault mechanics, and could constitute an interesting research avenue in the future.
Such dialogue between scales (following, for example, the main guidelines presented in this section) will be necessary in order to elucidate the complex interactions between confined sheared granular flows and their environment. The compliance of the two walls represented in Fig. 1, for example, is highly interesting in the frameworks of tribology and of fault mechanics because it is prone to control the scale of heterogeneities along the contact interface or along the fault. This includes heterogeneities in stress, in sliding velocity, in third body thickness, etc. By modifying the stiffness, we can imagine covering the whole spectrum of loading conditions, from the very compliant (no spatial correlation of the tangential motion along the interface) to the very stiff (infinite spatial correlation of the tangential motion). This variability is likely, for example, to strongly affect the scaling laws describing the statistics of sliding events in analogue seismic faults (46). The deformability of the first bodies is also a necessary condition for the dynamic triggering of sliding events, in close connection with the concepts of fracture mechanics, but with the added complexity of enriched friction laws arising from the lower scale. In future years, we can certainly expect a large number of studies in this area, since contact heterogeneity is often mentioned as an important feature (63) but rarely studied in detail.
iii . Computational aspects
Because of the experimental limitations exposed in section III.i, an important part of the future of studies on sheared confined flows dedicated to tribology and fault mechanics lies in numerical simulation. Obvious difficulties related to the breadth of the scales involved and to the associated computational costs have been covered in section III.ii. But they are not the only challenges to be expected in the numerical simulation of such granular systems. We can mention two major ways current numerical tools could be improved to increase our understanding of the physics and kinematics of these flows:
As mentioned in section II, the deformability of the grains is essential in all cases where the third body is submitted to large enough stress levels and is not prone to grain breakage. This includes, for example, tribological contacts in ductile materials such as metals or polymers, and fault gouges submitted to a temperature- and/or pressure-related brittle-ductile transition. As we pointed out, several simulation tools already exist for this task (24- 30), including the Multibody Meshfree Approach implemented in MELODY (33,34). Most of these methods, however, rely on a discretization of each grain, in order to provide much more kinematic freedom and to make its deformability possible, while coupling this deformability with the equations of continuum mechanics. This constraint considerably increases the computational cost of these methods when compared to classical DEM, because the number of degrees of freedom is typically multiplied by a factor ∼ 100 and the critical timestep of explicit solvers is reduced by a similar amount. Such methods can therefore handle, at most, about 102-103 grains, while current DEM simulations can typically deal with more than 105-106 grains. To overcome these limitations, the Soft Discrete Element Method (SDEM) was recently proposed (64). This novel approach relies on the fact that, to a first order, the physics of collections of deformable grains can be considered as fairly simple. When compared with rigid grains, two main phenomena seem to be primarily important. The first is the closure of the pore space, associated with the flattening of the grains around their contacts, and with the presence of contact segments/surfaces instead of quasi-point contacts (Fig. 5a). The second is the general ovalization of the grains when submitted to a deviatoric loading (Fig. 5b). The SDEM is based on two main ideas aiming at dealing with these two observations: large interpenetrations corrected by volumetric strain in the grains (Fig. 5c-e), and reduced kinematics based on rigid-body motion and on a homogeneous strain tensor in each grain. This simplification presents the interest of limiting the number of degrees of freedom (6 DOF per grain in the 2D case), and of keeping a rather large critical time step. It was shown in (64) that this method could reproduce fairly well some theoretical and numerical results in the case of the isotropic or oedometric compression of frictionless elastic discs. Thanks to its simplicity and versatility, it is likely that SDEM is prone to implementation in largescale optimized DEM codes, and we can expect it to be used in large scale simulations of collections of soft grains. The applications in tribology will be straightforward. Apart from the implementation effort we just mentioned, there are, however, several technical obstacles to overcome. More complex contact laws (e.g. friction, adhesion, etc.) and constitutive laws (e.g. plasticity, viscoelasticity, etc.) must be implemented, calibrated, and validated. The complexity lies in the fact that, since contacts involve deformation, a large part of the contact laws must be related to the constitutive laws of the material composing the grains. These complex topics are expected to be tackled in the near future.

Figure 5 Towards a less costly way to simulate soft grains (64). a: Snapshot of a Multibody Meshfree simulation of soft grains under isotropic compression, showing the contact flattening and the pore space closure; b: A similar simulation but with a deviatoric loading, showing the general ovalisation of the grains; c: The classical DEM point contact between discs with a sufficiently large contact stiffness; d: The SDEM segment-contact between ellipses with a large local deformation; e: Correction of the surface deficit associated with the large interpenetration through an additional term on the volumetric strain of the grain.
In the contexts of tribology and fault mechanics, a large effort must be undertaken to enrich the physics of such simulations. Such an effort was already undertaken by several authors (19,20,66,67), and needs to be pursued. This is the case, in particular, of thermal effects. As we mentioned in section II, MELODY can monitor the mechanical energy dissipation in each grain during a confined shear flow and compute the associated creation of thermal energy. At the moment, no heat diffusion has been implemented in the code, and this phenomenon is accounted for in an approximate manner, as a second pass on stored numerical results. Future versions of the code will need to natively handle thermo-mechanical coupling in order to deal “on the run” with (i) heat creation by interparticle and intraparticle anelastic phenomena, (ii) heat diffusion between grains and in the surrounding medium, and (iii) temperature-related changes in the rheology of each grain, either through its contact laws or through its constitutive behavior. This will enable improvements in our understanding of, for example, melt-related ultra-localization in fault gouge (40) or tribological flash-heating in the presence of a third body.
IV. Conclusion
This short paper presents an overview of some recent results regarding the simulation of confined sheared granular flows dedicated to applications in tribology and fault mechanics. After describing the recently observed complexity that can arise in such flows if some common assumptions (samples of quasi-monodisperse circular/spherical rigid grains) are partially or completely relaxed, we propose several research avenues that are likely to be tackled by the scientific community in the coming years. If sufficient effort and resources are dedicated to this task, we may expect a better understanding of tribological systems, including seismic fault systems, with possibly large scientific, environmental, economic, and human implications.