I. Introduction
The potential impacts of climate change on earth systems raise a major societal concern. Unexpected heat waves and heavy rains (1) occur more often in recent years, setting a new normal for the sustainable design of the natural and built environment. Extreme wetting/drying not only leads to crop yield reduction but also a decreased safety for the built infrastructure (5) (e.g., dikes and slopes). Soil, home to all plant species and many microbes, the material that almost all infrastructure is built under, is strongly connected with climate change. For instance, a large amount of soil organic carbon mobilization (e.g., erosion and tillage) contributes to atmospheric CO2 emissions. Meanwhile, the built infrastructure is being tested for its resilience against the expected impacts of climate change (e.g., floods and droughts). The concern for climate change-related risks calls for “nature-based solutions” (NBS) to make the built environment more sustainable (e.g., vegetated dike slopes (3)) and climate-neutral (e.g., storing carbon in the soil (6)). Understanding the bio-hydromechanical processes in vegetated soil is the prior knowledge essential to many of these nature-based solutions.
The vadose zone between the land surface and the groundwater contains complex soil composites that primarily consist of soil, plant roots, and pore water, together with microbes and other soilinhabiting animals. The soil microstructure there is constantly perturbed by the growth of plant roots. At the same time, root systems respond to the mechanical impedance of soil, leading to various types of root growths (e.g., seminal and lateral (7)). Furthermore, different components of a root system have specific functions, and the spatial distribution depends on the genetics of the plant and the type of architecture it produces. For example, monocotyledonous (fibrous) and dicotyledonous (taproot) are two main root system types (8, 9). The first system starts with the primary root probing the soil until other roots arise from the node (i.e., seminal, nodal, crown roots) and then uptake nutrients. The second system produces a first root, the so-called taproot, often reaching the greatest depth, with other basal roots growing from the node and the primary axis. Although the genetics of the plant is important, a key factor for a plant to secure resources is the topology and morphology of the root system (10). The availability of water in this vadose zone is crucial because root growth rate (e.g., elongation and branching) is a function of both mechanical and water stress. Nevertheless, studies on how the dynamics of root system growth and water uptake alter soil characteristics, as water retention and mechanical strength, are still limited.
It is well known that soil is a complex assemblage of mineral and organic particles that can host a very diverse range of biological organisms (11). To understand the scale of these biological activities, one needs to detect fluorescence markers, and methods such as MRI or X-ray CT do not have this ability. Transparent soil is mostly used to quantify the root length and diameter of each hierarchical order of the root architecture, and to capture cellular events.
For the macroscale observations, (12) developed a substrate called transparent soil, with a matrix of solid particles and a pore network containing liquid and air. The physical structure was manipulated with the aim of generating 3D optical images of soil biota in a physically complex yet controllable environment, mimicking the chemical and physical properties of the soil. Their results (12) show that root growth in transparent soil is similar to those quantified in agricultural soil. Concerning the microscale monitoring, an example is given by (13) that used transparent soil to image the 3D distribution of auxin in Arabidopsis thaliana root tips using auxin reporter lines.
The challenge is twofold: (1) to understand how different bio-hydro-mechanical processes are coordinated under extreme climate conditions and (2) to integrate the knowledge of fiber reinforcement, unsaturated soil, root-zone hydraulics, and rootvegetation growth to constitute a predictive, comprehensive description for vegetated soils.
Today’s climate and crop prediction models (4) are largely based on simplifying assumptions without considering the interactive processes between soil deformation, water uptake, and root growth at the microscopic scale. Developing a multidisciplinary understanding of these coupled processes is essential to improving existing climate and crop prediction models so that the estimation of carbon storage in agro-geotechnical systems can be more accurate.
Fig. 1 defines the topics relevant to the proposed challenge; the key focus is on the mechanical and hydrological reinforcement induced by growing roots. In Secs. II and III, the state-of-theart knowledge of underlying bio-mechanical and bio-hydrological processes in vegetated soil is reviewed. Root-soil-water systems constantly evolve under external wetting-drying cycles. This is the challenge to be addressed in Sec. IV. In Sec. IVi, we discuss the knowledge gaps to be bridged in order to unravel the interplay between soil deformation, root growth, and water/solute uptake. In Sec. IVii, we suggest a bottom-to-top approach and elaborate on the two main topics which the authors are currently investigating, including (1) the microscopic observation of rooted soil under drying-wetting via X-ray microtomography and (2) the coupled modeling of moisture transport, root growth, and soil deformation. A summary of this opinion paper and an outlook are given in Sec. V.
II. Mechanics of rooted soil
In the study of root-soil interaction, many factors are at play: the architecture of the root system, the soil heterogeneity and the pore structure, the pore water distribution that varies spatially and temporally due to evapotranspiration and root suction, and a combination of all these that alter both (the types of) root growth and soil matrix. In the following, we consider fiber-reinforced and partially saturated soils, i.e., the two limiting cases where water transport and root growth are absent. We review continuum theories that may shed light on the macroscopic modeling of rooted soil and then discuss the state-of-the-art experimental and numerical studies that provide microscopic insights into root-soil interaction.

Figure 2 Stress paths of the reinforced soil matrix and the root-fiber system as a whole (adapted from (14,20,21)). The bold line corresponds to the stress path of the fiber-reinforced soil matrix in comparison with a normal triaxial path indicated by the light straight line. Note, for the sake of brevity we only consider triaxial compression. Interested readers are referred to (15) for the stress paths of reinforced soil subject to uniaxial compression and simple shear, and (14) for a constitutive model for triaxial compression/extension.
i. Soil-fiber mixture: a simplistic analogy
Before addressing the biological aspects of rooted soil, let us consider cases where plants grow much slower than the variation of environmental loads and the induced soil deformation. In this limit, an analogy can be established, that is the fiberreinforced soil (14), either structured (geotextile sheets/containers) (15) or unstructured (fiber additives) (16). The inclusion of fibers introduces two reinforcement mechanisms, namely interlocking and confinement, that promote and sustain frictional resistance of the composite material as a whole (17-19). Recent studies (14,20,21) have revealed the underlying mechanism of fiber reinforcement, that is the stress state of the reinforced soil asymptotically approaches the failure surface, as shown in Fig. 2. A network of fibers may restrain sliding at the soil-fiber interface while elevating the confining pressure because of confined dilation. This interlocking-confinement mechanism provides further stabilization to the rooted soil, making it resilient to strong mechanical loads.
Utilizing the rule of mixtures, the upper-bound and lower-bound elastic properties can be found by adopting the volume-weighted sum formulation for the stresses or strains, namely where σ and ε are the total stress and strain tensors of the composite, ϕs and ϕr are the volume fraction of the soil and fiber phases, σs and σr are the stress borne by the soil matrix and root inclusions, and εs and εr the corresponding strains. Based on one of these stress/strain partitions (Voigt’s hypothesis or Reuss’s hypothesis), constitutive models for fiber-reinforced soils (14) are developed by plugging in the constitutive law of each constituent and assuming a certain level of sliding between the soil and fibers (22). Because of this close analogy, experimental and numerical approaches for soil-fiber mixtures can be conveniently extended for rooted soils (e.g., see (22-24)).
ii . Partially saturated soil
In addition to the mechanical reinforcement from roots or fibers, negative pore water pressure or suction between soil particles contributes to the total stress of partially saturated rooted soil. The theoretical framework for unsaturated soil mechanics (25-27) is well established and can be used as the basis to formulate the stress decomposition for wet rooted soil. For example, using Bishop’s effective stress formulation, the total stress of the soil matrix (including the pore water) σ s is extended as
where σ s ′ denotes the effective stress contributing from the contact forces between soil particles, p a and p w are the pore air pressure, including vapor and dry air, and pore water pressure, and χ ∈ (0,1) is the Bishop parameter. Note, χ = 0 and χ = 1 represent two limit cases, namely a completely dry soil and a fully saturated soil. For the intermediate degrees of water saturation s w ∈ (0,1), one could imagine capillary pressures in a network of waterinvaded pores effectively defined by the pore “diameters”, as it is usually done in tube-network models (28) based on the Laplace equation. Therefore, the first assumption one can make for the Bishop parameter is χ = s w . Nevertheless, the debate on the expressions for χ is still ongoing and requires microstructural considerations (29). Considering the effects of root structure and other physico-chemical interactions (30,31) (e.g., mucilage) at the soil-root interface, the effective stress theory for vegetated soils could be even more complicated. For example, the inclusion of roots could lead to anisotropy and heterogeneity in the soil microstructure, justifying the necessity for a tensorial Bishop parameter (32,33).
It is worth noting that, without considering root inclusion and micro-mechanisms on the stress partition, encouraging results were obtained for the numerical modeling of vegetated soil, as reported in Woodman et al. and Meijer et al. (24,34). iii Root-zone bioicromechanics
Current knowledge of root growth in partially saturated soil is largely based on the assumption that roots and the hosting soil behave as a continuum composite, which can be described by macroscopic variables such as Young’s modulus of the root system, soil penetration stress (e.g., confining hydrostatic stresses (35)), and pore water pressure (36). Basic assumptions like Eqs. (2) and (3) need to be revisited because of the evolving, highly heterogeneous, and interactive root structure of vegetated soil. The classical view of root growth biomechanics is challenged to predict morphologies and developmental patterns of root systems in heterogeneous soils. Classical continuum mechanics need to be revised in order to account for the complex soilroot interaction that originates from the interface between root branches and soil particles. In the following, we review recent experimental and numerical studies that are aimed at a better understanding of the root-zone bio-micromechanics from the particle/root scale.
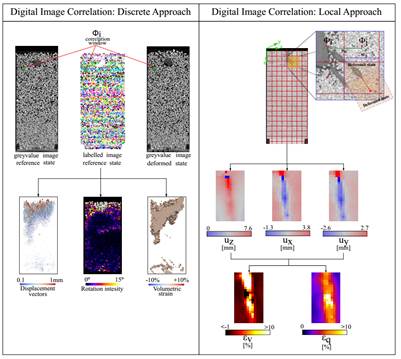
Figure 3 Sketch representing the discrete and local approaches typical of the digital image correlation used in root-soil interaction (adapted from).
a Soil kinematics and microstructural evolution due to root growth
In the past decades, X-ray microtomography has been revealed to be a relevant tool for observing the evolution of root expansion and elongation, in different types of soil, from sand (37), to sandy loam (38), and to vegetated soil (39,40). 3D imaging with X-ray microtomography has been widely used in the past decades: Table 1 presents an overview of these studies. In combination with X-ray tomography, Digital Image Correlation (DIC) is used to assess the spatial transformation of images (translation and/or distortion) between the reference state and the deformed one, which needs to be investigated. Such information can be either discrete or continuous, with the tracking of the single 3D object or gray level patterns in local sub-regions of the volume, respectively, as shown in Fig. 3. In the study of rooted soil, DIC provides the kinematic of the evolving interaction induced by root, revealing information on the response of soil to root growth, and water uptake.
The two most investigated soil properties affected by root growth are porosity and soil density. Both are usually quantified in the 3D space around the root surface based on either the binarized image (39) or the variation of greyscale (41). One of the first studies on this topic (42) suggested the compaction of soil around the growing root decreases exponentially with the lateral distance from the root. The model presented by Dexter is only in partial agreement with some more recent findings, such as the ones by Helliwell et al. (39), Koebernick et al. (43) and Lucas et al. (41). These authors observed a small zone of increased soil porosity close to the “flanks” of the root, generally attributed to a geometric effect due to the steric exclusion of soil particles near the root surface. Further from the root, no systematic trend was observed, and the soil was seen to either contract or dilate from an initial loose or dense state.
Recently, Anselmucci et al. (37) run a 3D analysis of the local porosity in the vegetated samples and conclude that the root growth increases the sand porosity in the vicinity of the root and the dilated zone’s characteristic length depends on the initial density of the soil, in agreement with previous literature. The strain fields show that roots induce both shear and dilation in the soil during growth (axial elongation and radial expansion). Therefore, it can be suggested that the fall-off trends of porosity described and confirmed by many authors are likely to be correlated to the shear induced by the root elongation, and not only due to steric exclusion as supported in previous research.
b Modeling root growth at the root-particle scale
X-ray microtomography provides a threedimensional, non-intrusive measurement of microstructural evolution in root-soil systems. The stress transfer and pore water distribution, however, are nearly impossible to obtain experimentally. Meanwhile, there is an increasing need to fundamentally understand how roots grow, displace, and stabilize between soil particles, largely motivated by a growing interest in bio-inspired robotics. With particle-scale modeling techniques, such as the discrete element method (DEM), mechanical interactions between particles and root branches can be simulated explicitly. The recently developed deformable DEM is a promising approach to model soft objects with the Minkowski sums of polytopes and spheres (16). In (16), the roots were modeled as chains of interconnected cylinders and the softness of the roots is offered by tensile, tangential, and bending/twisting springs set according to beam theory. The discrete cylinders can disconnect if the tensile/bending/twisting strength is overcome.
In addition to the complex topology, root systems are multifunctional, with branches and hairs contributing to anchorage, elongation, and penetration. These bio-physiological characteristics have recently been considered in DEM modeling: in a recent work, Fakih et al. extended the spheroline approach by assigning a constant growth rate at the root tip while stiffening the spheroline segments in regions further behind the tip. This allows a realistic representation of “old” root branches. Subjected to contact forces from soil particles, the root, modeled as a self-elongating spheroline, can effectively probe the pore space and maintain the deflections when turning from young to old. Nevertheless, a more complete DEM root model that takes into account the external stimuli (e.g. tropism) for root growth orientation and elongation rate is still rare.
III. Bio-hydrological processes in rooted soil
While geotechnical engineers are generally interested in the mechanical reinforcement effect of plant roots, the topic of (living) roots in the soil is treated more comprehensively by botanists, hydrologists, and plant scientists. For vegetation growth, the transport of soil moisture to the atmosphere, via the root, stem, and leaf, is crucial. Despite the different focuses, the relevant processes common in unsaturated soil mechanics and root-zone hydrology are (1) Darcy-type flow combined with mass balance (also known as Richard’s equation) and (2) (the hysteresis of) soil water retention. In the following, we review the moisture and heat transfer that has been widely investigated in recent years. The second aspect, that is the variation of soil water retention capability with respect to root growth, requires careful consideration and will be discussed in Sec. IV.
i . Coupled moisture and heat transport
The effects of soil moisture transport and temperature gradient on unsaturated soil mechanics have been studied extensively. In the computational hydrology community, the focus is on the transport of soil moisture due to water potential and/or temperature gradients, as these transport processes ensure water availability for plant growth via photosynthesis. The importance of soil moisture modeling has led to the development of numerical models that allow simulation of water transport, in both liquid and vapor phases. Despite wide applications (e.g., Hydrus,), these models treat the soil to be a rigid porous medium.
The governing equations are based on Philip and de Vries’ early work on the transfer of heat and moisture in porous media, first published in 1957 . Milly later extended the theoretical framework in 1982 to account for the hysteresis of water retention and implement the one-dimensional formulation in a finite element model. In essence, the governing equations consist in coupling Darcy’s law with mass conservation for the water and air phases and closing the continuity equations with constitutive laws. Assuming mass and heat flow in the vertical direction z is predominant, the total moisture balance can be simply expressed as
where ϕ L and ϕ V are the volume fraction of water in the liquid (L)) and vapor (V) states, ρ L and ρ V are the densities of water, q L and q V are the corresponding mass fluxes, and S(z,t) is the sink term (neglecting evaporation) for the root water uptake that varies in time t and also in space. The soil water potential at depth z ψ s(z,t) and the leaf water potential at the surface of the root ψ l(t) is what drives the water uptake. The proportionality α is essentially derived from the root architecture, e.g., by taking into account the axial conductivity and root geometry (see its various formulations in (34).
Substituting the following constitutive laws into Eq. (4) closes the mass balance equation for the moisture phase.
where K is the unsaturated hydraulic conductivity and can be computed from the Van Genuchten model that relates the saturation degree s w to K and the suction p a −p w (see (3)), D e is the molecular diffusivity of water vapor in soil, u is the pore water pressure, and γ w is the unit weight of water. Note, for demonstrative purposes, Eqs. (4)-(7) contain a minimal set of parameters for the moisture phase alone. To model simultaneous dry air and moisture flow through unsaturated porous media, the bulk flow of dry air and the dissolved dry air into pore water need to be considered. A more complete formulation with the energy balance coupled to the air (including both dry air and vapor) and liquid water transfer in unsaturated soils as a three-phase system can be found in.
In soil mechanics models where the medium’s irrecoverable/inelastic response to stress is the primary focus, water vapor transport is usually neglected. Indeed, moisture transfer under temperature gradients is negligible in fully dry and wet conditions, but it becomes relevant with a fairly welldefined maximum at an intermediate moisture content, depending on soil-water tension and air-filled pore space. Meanwhile, soil microstructural changes due to repeated wetting-drying cycles and root penetration can make classical constitutive relations inadequate. For example, diffusion in granular materials that consist of polydisperse or irregular-shaped particles is found to be nonFickian; the hydraulic conductivity has strong dependencies on the soil microstructure, leading to non-Darcy flows.
ii. Coordination between root hydraulics and root growth
In the previous section, we see that, from a macroscopic perspective, the uptake of soil water can be described by a sink term S(z,t), which can be quantified through homogenization of the radial and axial flows from soil to the roots, via the xylem. Meanwhile, root growth depends on external stimuli such as water content and mechanical impedance; the ability of root systems to adapt to these spatially and temporally varying stimuli is essential to the efficient acquisition of water and nutrients. For example, a deep rooting system reduces nitrogen losses through leaching and improves drought tolerance, while shallow rooting is important for the acquisition of immobile resources such as phosphorus (P) (see for a comprehensive review). The underlying hydro-biomechanical processes that coordinate root growth and water/nutrient uptake, however, are not usually accessible. Complementary to microscopic observation (e.g., X-ray CT) are numerical simulations that take into account the coupling between root growth and root-zone hydraulics.
Two families of numerical models exist to describe dynamic root growth in a soil matrix: (1) field density-based continuum models and (2) root system architecture-based discrete models. While the former relies on the parameterization of root system development as time-evolving probability density functions of field variables, such as root length, the latter aims to describe the development of root system architecture in great detail (the order of roots, the branching points, the segment lengths). These multi-scale approaches share similarities with those for numerical modeling of granular materials. However, their predictive capability is improved by considering stochastic variables or processes, e.g., in root deflection and branching, specific to a certain species.
In order to describe the response of root growth to the soil environment (e.g., pore pressure, nutrient concentration, pore size distribution), root architecture models, are coupled with soil water flow models. At the root scale, the root water uptake is driven by the time-evolving distribution of the water potential gradients between soil and root. RSWMS solves the soil water which flows from the unsaturated bulk soil to the soil-root interfaces, and eventually transported to the leaves through the root system . The water uptake is coupled to the so-called Doussan equation which explicitly solves the water flow in a root system given its three-dimensional architecture. One could utilize a homogenization approach where the complex root architecture and heterogeneous conductance is simplified as some spatial distribution functions of root length, root surface, and root mass densities, in a similar fashion as the fiber reinforcement to non-vegetated soil (see Sec. IIi).
IV. Root-soil-water interaction
In Secs. II and III, we reviewed theories and numerical models that separately describe the mechanical and bio-hydrological responses of rooted soil. In the following, knowledge gaps and current challenges that are crucial to the understanding of the rootsoil-water system as a whole are elaborated. Based on the main gaps and challenges, we propose an integrated, bottom-to-top approach to advance the multi-scale modeling of root-soil-water interaction. We elaborate further on ongoing experimental and numerical studies and explain how they may contribute to the development of next-generation soilplant-atmosphere models.
i. Main gaps and challenges
a Multiple time scales
Several time scales exist when describing plant growth in soil, such as the time scales for root water uptake (83) and root growth (84). In addition to these internal time scales, the time scale of the external water cycles must be considered, because of their high relevance to root growth. Depending on the growth stage, micro-meteorological conditions, and the availability of resources, these relevant time scales may vary significantly. For example, for very young plants, the rate of root growth and root water uptake are correlated (85). However, they may differ during a quick growth stage (86,87) and/or after fertilizer application or after the plant reaches maturity. It is noted that water deficit plays a significant role in determining the root growth and root water uptake and their variability in depth (see Figs. 2 and 6 in (88)).
When the underlying biological and mechanical processes (e.g., root water uptake and soil deformation) occur at very different time scales, theoretical or numerical models can be largely simplified, for example, by treating the soil as a rigid medium when the soil moisture is transported (Sec. III) or assuming the root system to be non-growing (Sec. II) during a rapid collapse of vegetated slopes. In a generalized framework, for environmental loads that induce bio-mechanical responses at comparable time scales, root-soil-water interaction must be taken into account. An example of this is the coupling of root growth, water uptake, and moisture transport in the bulk soil. In Sec. III, the problem has been simplified by treating the soil as a rigid porous medium. The response of a rooted soil to external environmental loads is certainly more complex when the root growth rate is comparable to the wetting-drying and/or freezing- thawing rates. Advanced 3D imaging allows for accessing the root-scale data and quantifying the relevant time scales, for certain species (37). The main challenge is how to generalize these time scales to the field where spatial effects (e.g., the influence of neighboring plants on each other) play a role.
b Effects of root growth on root-zone hydrology
Another fundamental characteristic of rooted soil is the water retention capacity. The soil-water retention surface is empirically built to relate water content, suction, and other state variables such as void ratio. When the soil undergoes wetting-drying cycles, hysteretic behavior (see Fig. 4) arises as a result of the collective effect of pore space nonuniformity, microstructural changes, among other microphysical processes such as air entrapment and capillary condensation. The Van Genuchten equation (67) is one of the widely used models to describe the wetting-drying behavior of unsaturated soil. However, the Van Genuchten equation is an empirical model fitted to experimental data and is therefore subject to the change of material compositions and inclusions, such as fibers and roots. To what extent the presence and architecture of root systems and the rate of root water uptake affect the soil water retention capacity of rooted soil remains unanswered.
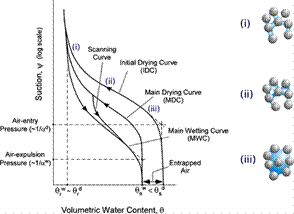
Figure 4 Hysteresis of soil water retention curves under varying saturation conditions, i.e., i-iii. (adapted from (89)). The mechanisms behind this hysteretic response include the ink-bottle effect, homogeneity in the interconnected pore network, the change of the soil microstructure induced by wetting or drying, etc.
In extreme cases where both root growth and water uptake are suppressed (e.g., by cutting off the leaves and sealing the stem), the root-soilwater system can be considered closed during wetting/drying, and the water retention capacity is mainly affected by adding the root networks to the soil’s contact network. In these cases, drying and wetting paths can be easily depicted, experimentally or numerically. However, being inert does not remove the possibility of moisture transfer between roots and the bulk soil, leading to shrinkage or swelling of the root tissues. Even when the water content in the root system is very small (e.g., for very young maizes), the migration of water content via the root network to the soil (i.e., hydraulic redistribution (90)) can still affect the water retention capacity. In extremely dry conditions, waterstressed roots will eventually shrink and lose contact with the soil, leaving gaps/channels for the water to flow through (91).
When root growth is considerable during wetting (and drying), both the moisture transfer and mechanical disturbance are enhanced. Root growth displaces soil particles, leading to irreversible changes to the soil microstructure and thereby very different drying and wetting paths. Systematic studies with and without considering a dynamic root growth are needed to extend existing soil water retention models for rooted soils.
c Effects of root growth on root-zone mechanics
Root growth and swelling or shrinkage during repeated wetting and drying can affect the mechanical properties of the soil matrix significantly. Unlike artificial fibers and geotextiles, root systems provide an active anchorage effect due to two components: 1. global anchorage due to the spatial extension of the racinary system; 2. local anchorage due to root hairs, which propagates in wider areas far from the main root system body and anchor to the pore walls (92). Additionally, roots have non-uniformly distributed mechanical properties depending on their age, and the hydraulic states of the soil plays an important role (93). Many theoretical studies assume a simple stress partitioning based on the volume fractions of the root, soil, and water phases (34). Questions regarding whether or not and to what extent the stress partitioning of partially saturated rooted soil depends on the (evolution of) root system architecture (topology) require investigations at the particle-root scale.
Promising constitutive models (23,24) have been proposed for rooted soil with predefined root system architecture. To develop mechanical models that can be integrated into a so-called soil-plantatmosphere framework (94), root system growth and its influence on the water retention capacity should be incorporated. In a similar fashion as the discrete and the continuum model are developed for the root-zone bio-hydrology, constitutive laws for rooted soil should be developed at the particle/root scale and the macroscopic scale, in order to facilitate their coupling. A multi-scale approach, from the scale of a single plant to the field scale via computational techniques such as homogenization (95) seems to be well suited.
ii . An integrated, bottom-to-top approach
The success of developing a coupled numerical model for root-soil-water interaction requires a synergy of multidisciplinary knowledge and tools. Because most bio-hydro-mechanical processes in the vadose zone are not directly or only partially observable, a combination of numerical modeling and laboratory/field testing must be adopted (69). Due to the complex composition and the evolving microstructure of root-soil systems, we propose an integrated, bottom-to-top approach, from the root/particle to the single plant and field scale. In the following, we summarize the proposed approach and steps to take. Possible roads to take at each step are discussed based on existing literature. In Sec. IViia and b, we elaborate on the two main topics (step 1 and 4) which the authors are currently investigating.
1 Relevant time scales: Perform wetting-drying experiments to obtain the time evolution of soil deformation and water content induced by the root growth and water uptake (see Sec. IViia). Via Xray CT, the spatial variation of soil deformation and water (re)distribution during wetting and drying can be quantified at the root scale. The relevant time scales estimated with X-ray microtomography and the moisture and matric potential sensors would elucidate to what extent the bio-hydromechanical responses need to be coupled and determine the level of details to be incorporated in the numerical model.
2 Stress formulation: Clarify the contribution of root reinforcement to the classical unsaturated soil mechanics framework, including stress partitioning between roots and the soil, constitutive laws at the representative volume scale, and their dependencies on the stochastic evolution of the root system architecture during growth. At the particle/root scale, how the stochastic and nonuniform characteristics of root system architecture affect the rootzone mechanics can be easily investigated, and is a necessary step to integrate the knowledge about root growth from the plant science community. a mechanistic framework is currently being developed by combining RSWMS (75) for root-zone biohydrology and YADE (96) for unsaturated fiber-soil mixtures. A coupling between RSWMS and YADE, as illustrated in Fig. 5, will allow performing numerical experiments when certain laboratory conditions are difficult to realize, providing data complementary to the X-ray microscopic observation.
3 Coupled root-zone bio-hydro-mechanics: Develop root/particle-scale numerical models, capable of simulating root growth, water uptake and transport in the soil, and their interaction with soil deformation, by coupling a root architecture and a discrete soil mechanics model. For example, a mechanistic framework is currently being developed by combining RSWMS (75) for root-zone biohydrology and YADE (96) for unsaturated fber-soil mixtures. A coupling between RSWMS and YADE, as illustrated in Fig. 5, will allow performing numerical experiments when certain laboratory conditions are difcult to realize, providing data complementary to the X-ray microscopic observation.
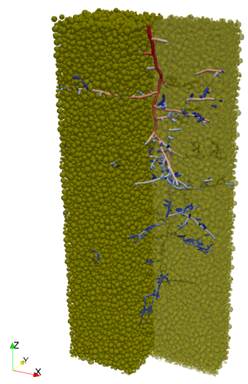
Figure 5 A coupling between root-zone hydrology and unsaturated soil mechanics based on detailed representations of root system architecture and soil microstructure.
4. Extend soil water retention: Extend the state space of the soil water retention capacity and hydraulic conductivity with root growth. A combination of laboratory and numerical experiments will help decouple the biological and hydro-mechanical responses of rooted soil, see Sec. IViib. The new mechanistic framework for the mechanics of rooted soil (Step 2) and the improved soil water retention models (Step 4) need to be coupled with the moisture, air, and heat transfer equations that describe the so-called root-soil-atmosphere “continand the pore water within the pores at different water contents and compare the same quantification for the base cases where the roots are absent. The soil samples are prepared in cylindrical polymethylmethacrylate (PMMA) containers of 3cm inner diameter and 10cm height, as illustrated in Fig. 6a. The samples consist of partially saturated sand (D50 = 400/600µm) accommodating a living three-day-old maize plant . A thin sheet of paraffin will seal the soil-free surface (excluding the stem) to keep the water content (W c ) constant during the scan, while the bottom is a 3D printed base designed to allow water infiltration.
The samples are dried and watered - or vice versa - within an established global water content range (5% ≤ W c ≤ 30%), chosen to avoid significant water stress during the root growth stage. Readings of water content and matric potential are continuously obtained during the imposed drying and wetting paths. We select three water contents (6%, 12%, 18%) at which the X-ray imaging is conducted. Between two consecutive scans, the samples remain in a growth chamber where the temperature and humidity are kept constant, while water content and matric potential are recorded continuously. We repeat each experiment three times in order to ascertain that the experimental data are reproducible and representative, taking into account the aleatory behavior of living plants. In parallel, two non-vegetated samples are prepared, monitored, and scanned, following the same drying-towetting and wetting-to-drying paths. Experimental data obtained from the non-vegetated and vegetated samples are compared to see how soil microstructure and pore water distribution are affected by the root water uptake and growth during wetting-drying cycles. Fig. 6b-c demonstrate the detection of the soil, root, and water phases within the specimen, with the aid of novel image processing tools developed in (54). The goal is to extract the 3D microstructure and the water interlocked within the pores at different water contents and compare the results between vegetated and unvegetated media. Taking into account the stochastic behavior of living plants, the experiments are repeated three times. Fig. 6c illustrates the expected output from the image analysis.
b Extending the soil-water retention surface with root growth
In the above-mentioned experiments, a transient process is monitored where roots continuously grow and affect the soil microstructure. Although a transient response is probably more realistic, the time scales of root growth and drying/wetting are not easily separated (see the discussion in Sec. IVia). The wetting-drying conditions may not be precisely imposed because part of the soil water is lost through transpiration and used by plant growth. In order to avoid the interdependence between plant or root growth and wetting-drying, we suggest an integrated approach combining numerical and laboratory experiments.
Physical experiments: Depicting the water retention surface for vegetated soil with the roots growing is a challenging task. If the leaves are trimmed off, as illustrated by the intermediate steps in Fig. 7, the root system can be assumed to be inert, excluding the influence of growing roots on the water retention capacity. To achieve this experimentally, we grow the same plant species in different samples and remove the above-ground part at different dates of their growth. The evaporation/imbibition process provides a good estimation of the drying/wetting branch for a given state of root growth and soil microstructure. From these drying/wetting experiments, we would be able to understand the soil-water-retention surface shift due to the different content of bio-mass or root length density (see the dark to light green curves in Fig. 7).
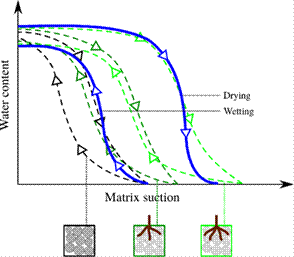
Figure 7 Illustration of soil water retention curves at various stages of the root system development (dark green to light green). The blue path exemplifies a transient behavior where the root growth dynamics is active.
Numerical experiments: In situations where the root system development is limited by the sample size, or the drying/wetting process cannot be precisely controlled, numerical experiments can be considered as an alternative. A computational model (see Fig. 5) can be calibrated using the 3D imaging data obtained from transient experiments (Sec. IViia) and root growth data from existing databases. In a virtual experiment, the influence of root growth on the soil water retention and induced soil deformation can be systematically studied by switching on and off root growth in order to obtain the transient and steady-state hydro-mechanical response. The stochastic behavior of root growth, which is one of the built-in functionalities of most root architecture models, can be utilized to extend the same analysis to different root types.
The root/particle scale data, whether experimental or numerical, can be homogenized over the sample volume in order to parameterize the soil water retention surface in a state space that consists of saturation degree, soil deformation, and root growth. Fig. 7 illustrates how the soil water retention curve can be parameterized with root growth and how experimental data may appear as transient response, depending on the root growth rate and the induced soil microstructural change.
c Integrating below- and above-ground data
Precise measurements of root morphological parameters, such as root length and mass densities, through time and space, are crucial to many hydrological models (97). Although 3D root-soil data are becoming increasingly available via X-ray microtomography (see Table 1), they are often limited by low throughput. High-resolution remote sensing of above-ground vegetation attributes is a promising approach to estimating the spatial profile of rooting systems from the single plant to the field scale. This indirect method is based on strong correlations between canopy structure parameters (e.g., above-ground biomass, volume, and leaf area index (LAI)) and root system patterns (98-100). Additionally, LAI is also well known as a key feature regulating plant transpiration, and thus strongly linked to root water uptake (101). Given the rapid development of 3D imaging technologies, 3D imaging sensors (e.g., digital cameras) mounted at various sensing platforms bring new opportunities for high spatial and temporal mapping of above-ground biomass and LAI at different scales (102,103). The plant growth data indirectly obtained from these remote sensing devices can be used to calibrate the (coupled) soil and root water transport models, creating a hybrid environment for continuous assessment of vegetation growth, moisture transport, and ground deformation.
V. Summary and outlook
Rooted soil represents a complex multi-phase granular system, with an internal mesoscopic structure that evolves via bio-mechanically coupled processes. The transport of water from the bulk soil’s pore space through the root system to the atmosphere provides one of the essential resources for the plant to grow. These coupled processes can become strongly influenced by the soil’s microstructural evolution, e.g., during extreme wetting and drying. In this opinion paper, the supporting processes of rooted soil for vegetation growth and their essential role in confronting the potential impacts of climate change, particularly drying/wetting, are reviewed.
Since the early theoretical work of Philip and de Vries (59) in the 1960s, there has been great progress across many scientific disciplines on different aspects of rooted soil. The root-zone hydrology is well studied with established governing equations for numerical models (61, 64) and advanced (remote) sensing techniques for obtaining laboratory and field monitoring data. The coupling between root-zone hydrology to root growth dynamics has been an active area of research in the last two decades (75). The main idea is to describe fluid flow within a root system architecture (80,81) and connect the fluxes between the root and the soil system (Sec. III). On the mechanics of (unsaturated) soil, theoretical frameworks have been developed as well (62). The long-standing questions of finding the microscopic origins (e.g., breakage, agglomeration, etc.) of macroscopic theories, or “upscaling”, have largely motivated the granular matter community. One of these microscopic origins is the mechanical reinforcement of artificial fiber inclusions (21,23, which shares great similarity with that of root systems (Sec. i).
Although studies dedicated to the coupling between root growth, water transport and uptake by roots, and soil deformation are still limited, the relevant technologies have been developed but are only scattered across different disciplines. Based on an extensive review of root growth-coupled mechanics and hydraulics, the main knowledge gaps are identified.
Firstly, the relevant rates (e.g., growth and water uptake) in comparison with the drying/wetting rates need to be quantified, before being able to determine the complexity of a coupled theoretical or numerical model.
Secondly, existing models of soil water retention curves need to be extended with root growth (dynamics) and an upscaling/homogenization of root architecture.
Thirdly, constitutive models for rooted soils should be developed based on a realistic physiological representation of root systems and the underlying assumptions revisited, adopting a multi-scale approach.
This opinion paper provides a comprehensive review and suggests roads for studying soil-waterroot interaction. We explain how emerging experimental and numerical techniques, namely, Xray computed tomography and particle/root-scale simulations could be utilized to bridge the abovementioned knowledge gaps.
Addressing the knowledge gaps and challenges presented here requires several research communities (e.g., physics, soil mechanics, hydrologists, and plant scientists) to work together. A community effort is being made via international workshops and schools, such as the ALERT Workshop “The mechanics of root-soil systems: from microscopic to macroscopic approaches” coordinated by Evelyne Kolb (PMMH & Sorbonne Universit´e) and Luc Sibille (3SR & Universit´e Grenoble Alpes) and the 12th ALERT Olek Zienkiewicz School “Looking into the rhizosphere: the interface between plant science and soil mechanics“ by Alessandro Tarantino (University of Strathclyde) and Enrique Romero (Universitat Polit`ecnica de Catalunya). These research activities create the knowledge base for educating young researchers about root-soilwater interaction and initiate collaborations beyond their communities.
To build a predictive framework for a fully functional vegetated or agricultural soil, the solute transport and uptake by roots and energy balance need to be taken into account, in addition to soilwater-root interaction. More importantly, together with plants, the microbial communities in the soil are essential to the stabilization of soil carbon, from the scale of a single plant to green infrastructure. Incorporating the biogeochemical processes would require an even broader collaboration beyond those mentioned above and these collaborations, workshops, and schools would give rise to a new interdisciplinary field that provides the essential knowledge and tools for the better design of the natural and built environment.
Acknowledgements - The authors would like to acknowledge the support from the Sectorplan B`eta Techniek of the Dutch Government.